Thank you for visiting nature.com. You are using a browser version with limited support for CSS. To obtain the best experience, we recommend you use a more up to date browser (or turn off compatibility mode in Internet Explorer). In the meantime, to ensure continued support, we are displaying the site without styles and JavaScript.
Scientific Reports volume 15, Article number: 3030 (2025 ) Cite this article Nature Slideshow

Microbial-induced calcite precipitation (MICP), which leverages ureolytic microorganisms, has received significant attention during the past decade as a promising method for sustainable building and geoenvironmental applications. However, transitioning from lab-scale experimentation to volumes suitable for practical use poses challenges. This study addresses these obstacles by screening and analyzing over 50 strains sourced from (i) a natural environment in the canton of Ticino in Switzerland; (ii) microorganism banks; and (iii) an industry-scale bioreactor. Several ureolytic Sporosarcina species have been identified in the natural environment, and their ureolytic potential has been compared with that of other strains. A reference, banked microorganism yielded the highest ureolysis rate. When this latter strain was inoculated in 900 L batches and continuously cultivated at 5400 L, no contamination issues were observed, and the reference strain remained the dominant species. The produced culture, obtained under an optimized medium composition involving the circular valorization of NH4+, was subsequently used to induce the biocementation of a 650 kg column of 0–1 mm sand. The results reveal the successful stabilization of the whole mass, with undrained Tresca strength values ranging from 90 to 140 kPa. This research lays the groundwork for scalable MICP production, which is capable of meeting the demands of real-world building and geoenvironmental projects.
There is extensive knowledge concerning the conditions and substrates that favor the growth of ureolytic strains and the subsequent precipitation of CaCO3 minerals. This process, widely known as Microbial-induced calcite precipitation MICP, results in the formation of mineral links that act as binders and strengthen the properties of soil aggregates1,2,3,4. These binders have been described in the literature as sustainable alternatives to commonly applied binders such as lime5 or cementitious grouts6,7, which are known to induce highly alkaline environments. Other binders, such as polyurethane resins and foams result in the release of potentially harmful substances upon degradation8.
MICP has been reported in several applications in the fields of geotechnical engineering9 and geoenvironmental work with a focus on erosion10, architectural structures and monuments11,12, carbon sequestration13 or even heavy metal and contaminant immobilization 14,15 and space exploration conceptual studies16. Notably, improvements in the mechanical17 or thermal properties18 do not compensate for the soil permeability because the pore space remains largely open17,19. A common denominator in all these works is the relatively attractive idea of using micrometer-scale agents, i.e., microorganisms that are native to various hydrogeological environments, and provide suitable conditions to induce the precipitation of CaCO3 in pH environments that are compatible with those of soils during a reaction which lasts several hours20. Among the most predominant ureolytic strains used are Sporosarcina pasteurii21 (Fig. 1), Sporosarcina aquimarina22, Sporosarcina ureae23, and Bacillus subtilis24.
CaCO3 crystal precipitation and S. pasteurii cells. Inlet (a) shows the typical calcite phase with distinctive planar and cubic textural features; Inlet (b) illustrates epicellular precipitation of CaCO3 on rod-shaped cells of S. pasteurii, which remain encapsulated and hence are considered inactive, as they are deprived of nutrients.
Typical media used for growth include yeast extract, Tris-buffer and ammonium sulfate (ATCC 1185921. Urea, nutrient broth and calcium chloride are widely used to induce CaCO3 precipitation in environments where microorganisms have grown at sufficient biomass levels and have ideally attached to the aggregate surface to provide nucleation sites (Fig. 1). With the exception of urea and calcium chloride, which are widely available and find applications in agriculture or other industrial processes, the remaining components have considerable supply and economic constraints, which raises questions around the application of MICP at realistic scales. Even if alternative nutrients are to be used, further challenges related to maintaining conditions that enable the growth of ureolytic strains in large-volume reactors, including temperature, the aeriation ratio25, and competition with other species26, are needed. For such large-scale volumes, sterile conditions cannot be easily ensured without sophisticated equipment. This would imply intermediary steps and longer timeframes of potential MICP applications in a field, i.e., building works, that favor fast, relatively simple, and straightforward steps of execution.
To the best of the authors’ knowledge, minimal information is known27,28, about the continuous growth of microbial cultures in the order of thousands of litters used to induce MICP while addressing the problem of contamination and the quantification of the complete timeframe, from the inoculation of bacterial batches to the removal of unwanted nitrogen byproducts.
Based on the above information, this work aims to address the following considerations. If MICP aims to harness naturally occurring microorganisms, they must be sourced from natural environments. To do so, this work compares the performance of naturally isolated strains to those sourced from microorganism banks. How can one subsequently grow sufficient biomass to induce MICP using solutions on the order of thousands of liters that would be compatible with real-scale applications? This growth should occur while maintaining noncontaminated conditions and avoiding costly bench-scale procedures, which might hinder the scalability of the technique (sterile conditions, energy-intensive steps and high-purity nutrients). For this purpose, several batches of 900 L each were grown continuously, and the resulting culture was subsequently inoculated in a 1.5-meter column composed of fine aggregates. This setup aims to provide a reference example at a scale that can be considered representative of real building applications.
The most widely used strain in the laboratory on the bench scale is Sporosarcina pasteurii21, which was used in this study as the reference (wild type). Another strain with known ureolytic potential was supplied by the Culture Collection of Switzerland under the reference name nhaC (CCOS 1977) courtesy of Medusoil SA. Furthermore, the continuous growth of this latter strain under nonsterile conditions in 2 L and 1000 L reactors resulted in four additional strains. These isolates, namely, b1 and b2 from the 2 L reactors and p1 and p3 from the 900 L reactors, were used in this study and were compared with the performance of the banked microorganisms. The cultivation protocol is described in the Materials and Methods section. In addition to these six cultures, we further assessed more than fifty morphologically different strains isolated from ammonia-rich soil. All the isolates were screened for the presence of urease by growth on urea agar base plates, and those that yielded positive results were further analyzed according to the steps described below.
The isolates from the environment, nonsterile reactors and reference cultures were subsequently screened for their safety to filter out potentially pathogenic strains and for their urease activity. For safety concerns, the isolates characterized using MALDI-TOF as belonging to the Bacillus cereus group were further screened using PCR for the presence of genes encoding the enteroxins hemolysin BL, nonhemolytic enterotoxin, cytotoxin K and cereulide, namely, hbl, nhe, cytK and ces, respectively. The presence of one of these genes was considered the cause of exclusion. The excluded strains were not further processed (i.e., their urease activity data are not available in Table 1).
The isolates that passed the safety test were screened for urease activity, as urease activity is the primary indicator of the performance of the strain with respect to MICP applications. This is due to the fact that ureolysis is the first step in the MICP process and metabolic pathway, which ultimately leads to calcium carbonate precipitation.
In April 2023, a total of 4 soil samples (10 g each) were collected from an agricultural area known to be exposed to high urea concentrations due to animal activity in the Ticino region in Switzerland (45°51’02.5’’N, 8°59’48.5’’). Samples from reactors inoculated with booster substrate and 900 L of bioreactor substrate were taken.
The isolation procedure for the environmental samples was performed following the methodology described in 29. This involved suspending a known quantity of soil in enrichment broth and sequentially isolating it on a urea-based agar plate (urea agar (Base) acc. CHRISTENSEN, Millipore, Buchs, Switzerland). The colonies that successfully changed color to pinkish-red due to an increase in pH associated with urease activity were then further investigated. The liquid samples were directly isolated on a urea-based agar plate.
The occurrence of urea hydrolysis during the growth phase of the isolates was determined based on conductivity measurements over time in triplicate for each strain. In fact, the hydrolysis of urea releases ammonia ions, increasing the conductivity of the solution. The theoretical value of mS/cm calculated from the urea concentration30 is considered the theoretical maximum value as per the relationship: Urea hydrolyzed (mM) = Conductivity variation (mS/cm) x 11.11.
A 50 ml inoculum of SP_nhca was inoculated into each of the four 2 L containers, which were supplied at constant temperature using a heating plate together with agitation at 2000 rpm, as shown in Fig. 2. The strain grows in 10 g/L peptone and 20 g/L urea media, which represents a notable shift from bench-scale protocols that mobilize yeast extract, Trizma base and ammonium sulfate. A total of 8 L cultivated for 48 h was subsequently used as inoculum in 1000 L containers. The culture-to-air ratio was 9/10 which explains why a 1000 L container receives a 900 L liquid culture. An air pump is constantly used as a means of agitation and aeration at the bottom of the container. Samples were collected at various times to assess the evolution of hydrolysis using electrical conductivity measurements.
Schematic representation of the 2-step batch growth of S. pasteurii, including (a) an initial inoculum of 8 L where the strains were sampled and (b) a 1000 L reactor, with air supplied as the means of agitation and aeration as well as constant temperature maintenance at 30 °C. Created in BioRender. Terzis, D. (2024) https://BioRender.com/k66m363.
Infiltration occurred from the bottom of the column via a 25 mm thick tube and progressed toward the top of the column. The bacteria from batch #6 were left for 12 h to attach before an equimolar solution of urea-CaCl2 (1 M) was introduced. An extraction pump was used to direct the effluent solution toward the tube again for a total of 24 h in 3 daily steps of 8 h each. After the last cycle, the extracted ammonium-rich solution was treated following the process described in31 to revalorize ammonium into ammonium sulfate, a fertilizer extensively used in agriculture.
Several sources for obtaining ureolytic microorganisms have been used. Specifically, these sources include (i) strains with known ureolytic potential for application of MICP supplied from two microorganism banks; (ii) the strains resulting from enrichment in 900 L reactors and the booster solution; and (iii) isolates from a natural environment (Fig. 3).
In the Materials and Methods section, the process of comparing and shortlisting candidate environmental strains is described. The two shortlisted environmental isolates (i.e., e1, e6) were found to yield lower urease activity than the banked microorganisms; however, their activities remained comparable to those of the isolates from the continuous growth in the 900 L reactors. Both the environmental isolates and the isolates from the large-scale reactor were identified as S. pasteurii by sequencing and database matching, yielding an identity percentage of 85%. The characteristics of the isolates and their urease activity values are reported in Table 1.
Bench-scale analysis of the ureolytic potential of eight strains. (a) EC monitoring and kinetic parameter assessment, including (b) the maximum slope of the curve (rm) and (c) the maximum value at plateau (ym). The same letters in the conductivity kinetics columns indicate significant differences at P < 0.05 according to one-way ANOVA and Tukey’s post hoc analyses.
When conductivity was used as a proxy for ion content (NH4+)32, in our analyses, the best performing strain (nhaC) reached a plateau at approximately 70 mS/cm (Fig. 4). Raw data for conductivity, cell counts and urease are reported in the supplementary material (S1). We postulate that the difference between the measured conductivity plateau and the theoretical maximum value is attributed to partitioning in the gas phase. The conductivity evolution of the six separate cultures was investigated by assessing the following kinetic parameters: the maximum slope of the curve (rm) and the maximum value at plateau (ym) (Fig. 3 b, c). The best performing strains were the S.pasteurii wt and the nhaC isolate: both are in fact faster at hydrolyzing urease with the fastest release of H + ions, which corresponds to a rapidly increasing EC. Based on these results, the nhaC strain was chosen to grow in 900 L reactors for a total of six batches and for the subsequent production of a biocemented column.
Batches of 900 L are produced using the upscaled MICP production setup described in the Materials and Methods section. In total, six batches are produced, and the results are presented from the first (#1) to the last batch (#6). Batches #3 to #6 used a residual 50 L from the previous batch each. This implies that the first two batches rely solely on inoculum from the 8 L cultures, i.e., the booster step described in the Materials & Methods, whereas the following batches receive both the 8 L booster step and the 50 L substrate of the preceding batch.
Bioreactor-scale analysis of the growth of ureolytic species in 900 L batches. The same letters in the conductivity kinetics columns indicate significant differences at P < 0.05 according to one-way ANOVA and Tukey’s post hoc analyses.
The results revealed relatively slower starts for the first and second batches, which both resulted in steady-state conditions in terms of ECs of approximately 60–65 mS/cm, consistent with the laboratory-bench scale campaign presented in Fig. 3. However, maintaining a fresh inoculum of 50 L in the reactor to launch the next batch significantly improved the hydrolysis of the batch. The results shown in Fig. 4 were used to compare the maximum slope of the hydrolysis curve (rm) and the maximum value at plateau (ym).
The last cultured batch from the aforementioned series was then used to infiltrate a column with a height of 1.5 m and diameter of 0.5 m, followed by the continuous circulation of a urea-CaCl2 solution. The results revealed that after the removal of the top 1.2 m of the casing, the sand column remained largely intact in the absence of confinement, which was the result of biocementation. The strength distribution on the front face of the column was evaluated using a pocket penetrometer, following a 10 × 10 cm grid pattern (Fig. 5). Similar methods have been used in model experiments using MICP, as described in similar studies33. With the exception of the top 15 cm— farthest from the infiltration outlet and effluent collection point—the remainder of the column presented strength values ranging from 1.8 to 2.8 kg/cm². The use of an empirical correlation commonly used34 to estimate the undrained Tresca strength from the pocket penetrometer resistance yields values between 90 and140 kPa.
A sand column composed of 0–1 mm aggregates infiltrated via a 25 mm tube was used to apply a bottom-to-top flow path at 5 L/min. The resulting spectrum of strength (kg/cm2) from the surface of the column was assessed using a pocket penetrometer.
This work presents findings across various scales concerning the application of MICP. Starting from the very source of the metabolic pathway implicated in MICP, i.e., ureolytic microorganisms, the work sheds light on the ureolytic performance of naturally occurring microorganisms. Specifically, at the bench scale, eight species from three different sources—(i) microorganism banks; (ii) isolates from the continuous growth of the former in nonsterile, large-scale bioreactors; and (iii) from a natural environment—are analyzed. Here, the banked strains were the most prevalent. The continuous growth of the selected strain in the 900 L batches revealed a ureolysis process that reached a plateau in approximately 100 h. No sign of contamination was identified, as all strains present in the bioreactor after continuous growth were Sporosarcina pasteurii. The produced bacterial medium was used to infiltrate a sand column with a height of 1.5 m, followed by 3 cycles of biocementation. After 24 h of infiltration, the produced column is endowed with sufficient strength to sustain its weight and remain integrated in the absence of confinement. The analysis of the front façade of the column reveals undrained Tresca strength values between 90 and 140 kPa, which is sufficient to serve a variety of geotechnical and building applications. Overall, this work aims to bridge the knowledge obtained across different scales and establish a shift in the paradigm toward the large-scale application of MICP to address practical problems, introducing notions of scalability and circularity through the treatment of unwanted, ammonia-rich byproducts.
The data supporting the findings of this study are openly available and can be accessed upon reasonable request. Interested readers are encouraged to contact the corresponding author (dimitrios.terzis@epfl.ch) for further details.
Phillips, A. J. et al. Engineered applications of ureolytic biomineralization: A review. Biofouling 29 (6), 715–733 (2013).
Article CAS PubMed MATH Google Scholar
Terzis, D. & Laloui, L. A decade of progress and turning points in the understanding of bio-improved soils: A review. Geomech. Energy Environ., 19, 100116. (2019).
Mori, D. & Uday, K. V. A review on qualitative interaction among the parameters affecting ureolytic microbial-induced calcite precipitation. Environ. Earth Sci., 80(8), 329. (2021).
Article ADS CAS MATH Google Scholar
Harran, R., Terzis, D. & Laloui, L. Mechanics, modeling, and upscaling of biocemented soils: A review of breakthroughs and challenges. Int. J. Geomech., 23(9), 03123004. (2023).
Dash, S. K. & Hussain, M. Lime stabilization of soils: reappraisal. J. Mater. Civ. Eng. 24 (6), 707–714 (2012).
Article CAS MATH Google Scholar
Anagnostopoulos, C. A. Effect of different superplasticisers on the physical and mechanical properties of cement grouts. Constr. Build. Mater. 50, 162–168 (2014).
Dhandapani, B. P. & Mullapudi, R. S. Design and performance characteristics of cement grouted bituminous mixtures-a review. Constr. Build. Mater. 369, 130586 (2023).
Article CAS MATH Google Scholar
Pillich, M., Schilling, J., Bosetti, L. & Bardow, A. What to do with polyurethane waste? The environmental potential of chemically recycling polyurethane rigid foam. Green Chem. 26 (21), 10893–10906 (2024).
Almajed, A., Lateef, M. A., Moghal, A. A. B. & Lemboye, K. State-of-the-art review of the applicability and challenges of microbial-induced calcite precipitation (MICP) and enzyme-induced calcite precipitation (EICP) techniques for geotechnical and geoenvironmental applications. Crystals, 11(4), 370. (2021).
Wang, Y. et al. State-of-the-art Review of soil erosion Control by MICP and EICP Techniques: Problems, Applications, and Prospectsp.169016 (Science of the Total Environment, 2023).
Jain, S., Fang, C. & Achal, V. A critical review on microbial carbonate precipitation via denitrification process in building materials. Bioengineered 12 (1), 7529–7551 (2021).
Article PubMed PubMed Central MATH Google Scholar
Delegou, E. T. et al. Metagenomics of the built Cultural Heritage: Microbiota characterization of the Building materials of the Holy Aedicule of the Holy Sepulchre in Jerusalem. Sci. Cult., 8(2). (2022).
Bandyopadhyay, A. et al. Microbial repairing of concrete & its role in CO2 sequestration: A critical review. Beni-Suef Univ. J. Basic Appl. Sci., 12(1), 7. (2023).
Article MathSciNet MATH Google Scholar
Rajasekar, A., Wilkinson, S. & Moy, C. K. MICP as a potential sustainable technique to treat or entrap contaminants in the natural environment: A review. Environ. Sci. Ecotechnol., 6, 100096. (2021).
Article CAS PubMed PubMed Central Google Scholar
Xue, Z. F., Cheng, W. C., Rahman, M. M., Wang, L. & Xie, Y. X. Immobilization of pb (II) by Bacillus megaterium-based microbial-induced phosphate precipitation (MIPP) considering bacterial phosphorolysis ability and Ca-mediated alleviation of lead toxicity. Environ. Pollut., p.124229. (2024).
Dikshit, R. et al. Space bricks: from LSS to machinable structures via MICP. Ceram. Int. 47 (10), 14892–14898 (2021).
Terzis, D. & Laloui, L. 3-D micro-architecture and mechanical response of soil cemented via microbial-induced calcite precipitation. Sci. Rep. 8 (1), 1416 (2018).
Article ADS PubMed PubMed Central MATH Google Scholar
Venuleo, S., Laloui, L., Terzis, D., Hueckel, T. & Hassan, M. Microbially induced calcite precipitation effect on soil thermal conductivity. Géotechnique Lett. 6 (1), 39–44 (2016).
Roy, N., Frost, JD & Terzis, D. 3-D Contact and Pore Network Analysis of MICP Cemented Sands. Grander matter, 25(4), 62. (2023).
Elmaloglou, A., Terzis, D., De Anna, P. & Laloui, L. Microfluidic study in a meter-long reactive path reveals how the medium’s structural heterogeneity shapes MICP-induced biocementation. Scientific Reports, 12(1), p.19553. (2022).
Yoon, J. H. et al. Sporosarcina aquimarina sp. nov., a bacterium isolated from seawater in Korea, and transfer of Bacillus globisporus (Larkin and Stokes 1967), Bacillus psychrophilus (Nakamura 1984) and Bacillus pasteurii (Chester 1898) to the genus Sporosarcina as Sporosarcina globispora comb. nov., Sporosarcina psychrophila comb. nov. and Sporosarcina pasteurii comb. nov., and emended description of th. Int. J. Syst. Evol. MicroBiol. 51 (3), 1079–1086 (2001).
Article CAS PubMed Google Scholar
Suresh, D. & Uday, K. V. A comparative study of various parameters influencing biocalcification via ureolysis mediated by enzyme and microbe: A comprehensive review. Geomicrobiol J. 41 (1), 17–34 (2024).
Article CAS MATH Google Scholar
Whitaker, J. M., Vanapalli, S. & Fortin, D. Improving the strength of sandy soils via ureolytic CaCO 3 solidification by Sporosarcina ureae. Biogeosciences 15 (14), 4367–4380 (2018).
Article ADS CAS Google Scholar
Feng, J., Chen, B., Sun, W. & Wang, Y. Microbial induced calcium carbonate precipitation study using Bacillus subtilis with application to self-healing concrete preparation and characterization. Constr. Build. Mater., 280, 122460. (2021).
Tang, C. S. et al. Factors Affecting the Performance of microbial-induced Carbonate Precipitation (MICP) Treated soil: A Review79 pp.1–23 (Environmental Earth Sciences, 2020).
Gat, D., Tsesarsky, M., Wahanon, A. & Ronen, Z. January. Ureolysis and MICP with model and native bacteria: implications for treatment strategies. In Geo-Congress 2014: Geo-Characterization and Modeling for Sustainability (pp. 1713–1720). (2014).
Omoregie, A. I., Palombo, E. A., Ong, D. E. & Nissom, P. M. A feasible scale-up production of Sporosarcina pasteurii using custom-built stirred tank reactor for in-situ soil biocementation. Biocatal. Agric. Biotechnol., 24, 101544. (2020).
Khosravi, M. et al. Large-Scale Bio-Cementation Test to Improve Sub-Structures of Existing Asphalt and Concrete Roadways. In Geo-Congress 2024 (pp. 135–144). (2024).
Bibi, S., Oualha, M., Ashfaq, M. Y., Muhannad, T., Suleiman & Nabil Zouari Isolation, differentiation and Biodiversity of Ureolytic Bacteria of Qatari Soil and their potential in Microbially Induced Calcite Precipitation (MICP) for soil stabilization. RSC Adv. 8 (11), 5854–5863. https://doi.org/10.1039/c7ra12758h (2018).
Article ADS CAS PubMed PubMed Central Google Scholar
Whiffin, V. S. Microbial CaCO3 precipitation for the production of biocement (Doctoral dissertation, Murdoch University). (2004).
Terzis, D., Laloui, L., Polytechnique, E. & Federale de Lausanne, E. P. F. L. Method and system for producing a carbonate-containing species-rich, nitrogen-containing species-free solution. U S Patent Application (2023). 17/925,960.
Krishnan, V., Tirkolaei, H. K. & Edward KavazanjianJr An improved method for determining urease activity from electrical conductivity measurements. ACS Omega. 8 (15), 13791–13798 (2023).
Article CAS PubMed PubMed Central Google Scholar
Xiao, Y. et al. Toe-bearing capacity of precast concrete piles through biogrouting improvement. J. Geotech. Geoenviron. Eng., 146(12), 06020026. (2020).
Holtz, R. D., Kovacs, W. D. & Sheahan, T. C. An Introduction to Geotechnical EngineeringVol. 733 (Prentice-hall, 1981).
The authors express their sincere thanks to the Swiss Innovation Agency (Innosuisse) for their support through the innovation project Ref: 02.649 IP-ENGMiBOC.
Faculty of Environment, Architecture and Civil Engineering (ENAC), Swiss Federal Institute of Technology, Lausanne (EPFL), Lausanne, Switzerland
Environmental Biotechnology, Institute of Microbiology, Department of Environment, Construction and Design, University of Applied Sciences and Arts of Southern Switzerland (SUPSI), 3 Medusoil SA, EPFL Innovation Park Building A, Manno, Switzerland
Camilla Perego, Margherita Cappa, Elisa Plant, Federica Mauri & Pamela Principi
Medusoil Sa, EPFL Innovation Park Building a, Lausanne, 1015, Switzerland
You can also search for this author in PubMed Google Scholar
You can also search for this author in PubMed Google Scholar
You can also search for this author in PubMed Google Scholar
You can also search for this author in PubMed Google Scholar
You can also search for this author in PubMed Google Scholar
You can also search for this author in PubMed Google Scholar
All authors contributed to the design and execution of the experiments presented in this manuscript, as well as to the interpretation of the results. Each author reviewed and approved the final manuscript prior to submission.
The authors declare no competing interests.
Springer Nature remains neutral with regard to jurisdictional claims in published maps and institutional affiliations.
Below is the link to the electronic supplementary material.
Open Access This article is licensed under a Creative Commons Attribution 4.0 International License, which permits use, sharing, adaptation, distribution and reproduction in any medium or format, as long as you give appropriate credit to the original author(s) and the source, provide a link to the Creative Commons licence, and indicate if changes were made. The images or other third party material in this article are included in the article’s Creative Commons licence, unless indicated otherwise in a credit line to the material. If material is not included in the article’s Creative Commons licence and your intended use is not permitted by statutory regulation or exceeds the permitted use, you will need to obtain permission directly from the copyright holder. To view a copy of this licence, visit http://creativecommons.org/licenses/by/4.0/.
Terzis, D., Perego, C., Cappa, M. et al. Biocementation beyond the Petri dish, scaling up to 900 L batches and a meter-scale column. Sci Rep 15, 3030 (2025). https://doi.org/10.1038/s41598-025-87074-9
DOI: https://doi.org/10.1038/s41598-025-87074-9
Anyone you share the following link with will be able to read this content:
Sorry, a shareable link is not currently available for this article.
Provided by the Springer Nature SharedIt content-sharing initiative
Scientific Reports (Sci Rep) ISSN 2045-2322 (online)
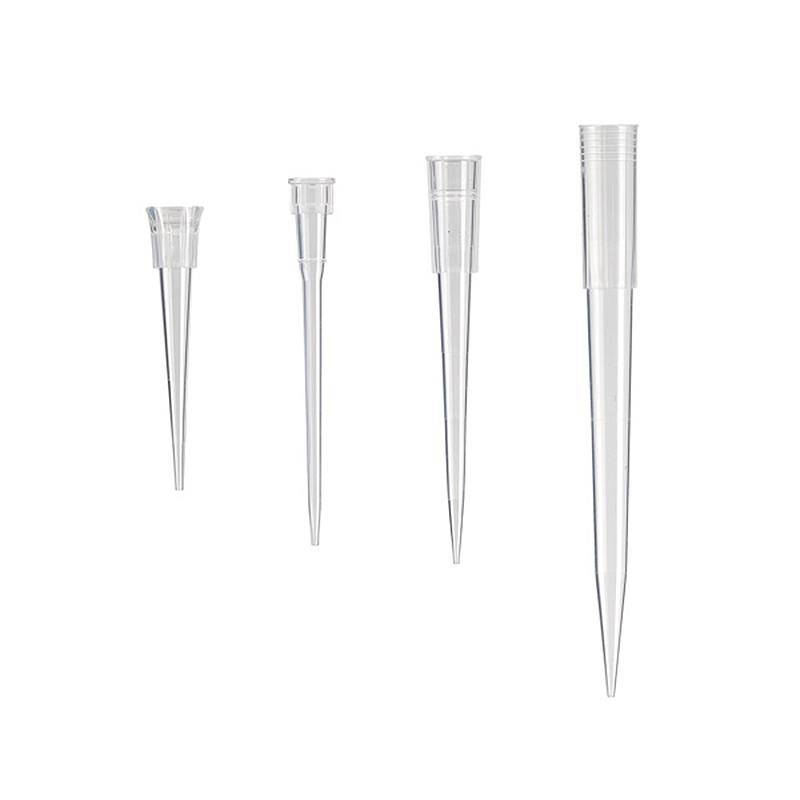
Slide Microscope Sign up for the Nature Briefing: Microbiology newsletter — what matters in microbiology research, free to your inbox weekly.